Romancing the Phantom Particle
- Julien Masbou, Astrophysicist
- 19 nov. 2018
- 11 min de lecture
The international Xenon project is the most sensitive experiment in the world devoted to hunting WIMPs. Buried under the Italian Gran Sasso mountain, Xenon1T has just completed a 278-day measurement campaign. While nothing was detected, it did refine the contours of this massive but-as-yet-unknown particle.
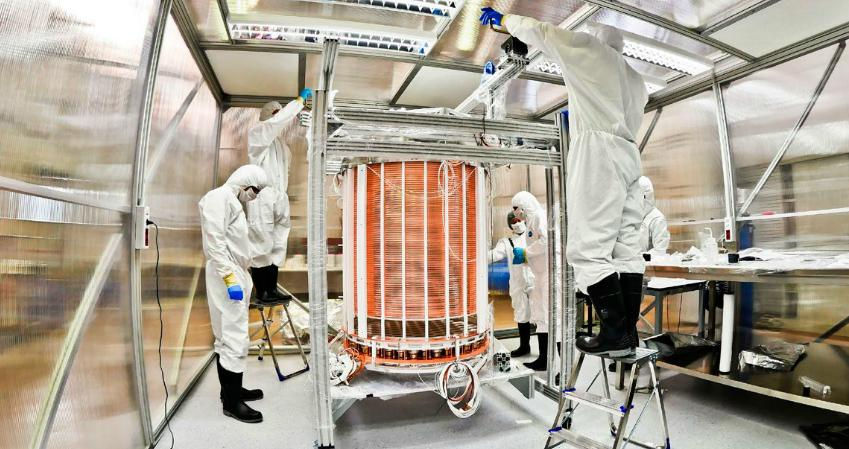
The heart of the Wimp detector named Xenon 1T, here assembled in a clean room, contains 2 tons of ultra-pure xenon at -100 ° C.
Photo © Xenon
Dark matter seems to be eluding physicists. From the depths of the Italian mountains to the Namibian desert, via Cern in Switzerland, the quest for it has engaged a large section of the particle physics community, like a giant treasure hunt. But they are still waiting to discover it after thirty years. Why do we persist in trying to observe this matter which is, by definition, invisible? Simply because there are so many arguments justifying its presence! The idea germinated in the 1930s in the mind of Fritz Zwicky, an astronomer studying the Coma cluster by analysing the distribution of speeds of some of its galaxies (see here). His conclusion was compelling: most of the mass of the Coma cluster does not emit light. Hence the name dark matter.
Although the measurement methods have changed, Zwicky’s results are still valid. In fact, it is quite startling to note how many methods there are to measure the mass of objects - kinetic measurement using galaxy rotation, or gravitational lens effect measurement - and how the conclusions remain identical; there seems to be five times more dark matter than baryonic (i.e. ordinary) matter. As well as measurements of mass, cosmological observations also support the existence of dark matter, mainly thanks to studies of the cosmic background radiation, emitted 380,000 years after the Big Bang. This radiation shows the universe as smooth and uniform, with only a few tiny fluctuations. Today however, 13 billion years later, the universe has become surprisingly imbalanced, filled with stars and galaxies separated by vast expanses of emptiness. How can this be so?
NEW PHYSICS
In what we call the “primordial soup” of the time of the Big Bang, the presence of a very massive, unknown particle, interacting weakly with ordinary matter, explains these observations simply. This particle should have a high mass to attract matter and form the first seeds of future galaxies. It should also interact weakly with ordinary matter, so as not to disturb the chemical balance in the early universe, in particular the proportion of light elements formed at the moment of the Big Bang, that we are able to measure with considerable accuracy. This is why the standard cosmological model, based on general relativity, now includes dark matter as one of its main ingredients.
Contrary to the infinitely large, the standard model of particle physics describes the infinitely small. Over the last few years, it has explained the interactions between particles, and has predicted new ones, since successfully discovered. In 2012, the discovery of the Higgs boson was a step forward in the understanding of our universe.
However, this success does not manage to explain several fundamental theoretical questions. For example, the mass of the electron is 511 keV/c^2, whereas that of the top quark is 170 GeV/c^2. Why does nature need particles with such different masses? Or, why is gravitational force 10^32 times weaker than the weak force, responsible for radioactivity only felt at short distances? To explain these observations, we need to arbitrarily adjust some parameters in the standard model. A more convincing alternative is to look for extensions to the standard model, designed to answer these issues; this is then referred to as new physics.
In particular, so-called supersymmetry models imagine super-particles that would interact in the same way as particles in the standard model, using the three main interactions - electromagnetic force, strong nuclear force and weak nuclear force. Their existence would solve numerous issues all at once; these three forces would be unified into a single force (usually imagined in modern physics) and, the lightest of these new particles could be the ingredient of dark matter, as it is supposedly neutral, stable and interacts weakly with the standard model particles. The candidates are usually called WIMPs, for Weakly Interacting Massive Particle.
INDIRECT APPROACH
Everything would seem to indicate the presence of dark matter particles in the universe. Other solutions are sometimes examined however, such as a modification to the theory of gravity. Alone however, it would have a hard time explaining everything so simply (see p.50). To detect dark matter, most physicists have been looking for the signs of its existence in the form of WIMPs since the 1980s.
Two very different approaches are used: direct detection intends to measure the passage of one of these unknown particles in a laboratory instrument, whereas indirect detection tries to gather “second-hand” traces, that would signal the existence of dark matter without catching the particle in question in its nets. Such traces are said to be produced when particles of dark matter collide and annihilate other. These cosmic events would produce particles of standard matter (protons, electrons, etc.) but also anti-particles, their opposite twins with an opposite electrical charge. These particles must be coming from space to us constantly; they can also result from known processes, such as the interaction of cosmic rays with their environment. Scientists are looking to detect an excess of these particles in the background noise caused by these known processes. From this point of view, the astrophysical phenomena generating antimatter are excellent candidates. All the more so as they are few and far between. If a notable excess of antimatter is detected, dark matter could therefore be easily suspected. The AMS-02 experiment, in the International Space Station in 2011, tracks these excesses of antimatter, while the Fermi satellite or the Hess ground telescope array in the Namibian desert, are looking for other excesses in gamma rays (*).
The work of the last few years has shown that the contribution of pulsars was greater than previously estimated. The resulting astrophysical background is far larger than we imagined, making the announcements of several detected excesses invalid. However, the excess recently observed by the American-Mexican telescope HAWC, sensitive to gamma radiation, cannot be explained by pulsars alone. Could this be the first indirect sign of dark matter? The debate among astronomers is bitter.
The advantage of the direct approach over the indirect approach lies in the fact that we control the detection chain far better in the laboratory. The principle consists of trying to observe the collision between a WIMP and the nucleus of an atom. The signal is very tenuous however. As a result, it is up to nuclear physics to create an environment conducive to its detection: a controlled environment in which the observed phenomena are more easily identified.
Direct detection is the only method that has the advantage of studying in the laboratory the dark matter particle that supposedly fills the universe. According to the speed of rotation of gases and stars, our galaxy, the Milky Way, is bathed in a halo of dark matter. The solar system passes through it at a speed of 220 km/s, our speed around the centre of the galaxy. By definition, a WIMP is a difficult particle to catch, but we believe that, every second, about 1 billion of them pass through 1 m² on Earth! Added to that is the 30 kph speed of rotation of the Earth around the sun, generating an annual variation in the flow of the order of 10%. It is precisely this annual variation that the Dama/Libra collaboration at the Gran Sasso national laboratory in Italy, claims to have detected. The interpretation of their result remains controversial (see inset).
Numerous teams have been trying for more than thirty years to catch sight of a WIMP. Some of the current experiments include LUX (United States), PandaX (Canada) or Deap (China), to name but a few. Today, the most sensitive dark matter direct detector in the world to look for the collision between a nucleon (**) and a WIMP is the international collaboration Xenon. It includes 165 scientists from 27 institutions in 11 different countries. This experiment which has been running for nearly twenty years at the Gran Sasso National Laboratory in Italy, uses liquid xenon. After two generations of instruments, the current experiment, Xenon 1T, uses 2 tonnes of xenon. It is the first dual-phase (liquid-gas) xenon detector in the world over one tonne. Xenon is a heavy nucleus which has the advantage of being naturally slightly radioactive. The heavier an element is, the more potential targets it offers to collide with dark matter: a nucleus of xenon has on average 131 nucleons.
Radioactivity represents a major problem in all these experiments as radioactive decay emits energetic particles that can also collide with the xenon atoms. Imagine a healthy, 70kg adult, regularly eating fruit and vegetables. They naturally contain radioactive elements, such as potassium 40. This person therefore emits 8,000 particles per second. Such flux would be too high in the detector, creating parasitic collisions, whereas today we are expecting dark matter to produce around one interaction (only!) per year and per tonne of exposed matter. In this type of experiment therefore, the “background noise” (all the events not resulting from the study object) must be eliminated. The background noise can come from three sources. Firstly, ambient radioactivity, which we eliminate using shielding, made of lead for example. Given its size, the Xenon project preferred to place its detector at the centre of a huge tank of ultra-pure water (10 m high and 10 m in diameter), which stops most of the radioactivity. Then, the detector itself is made of material that may be radioactive. The materials are carefully selected to limit and quantify this additional radioactivity.
ELIMINATING NOISE
Finally, cosmic rays from the highly energetic sun, supernovae or active galaxy cores for example, themselves accelerate particles that bombard the Earth regularly (20 particles/m²/s). To prevent them, the Xenon project set up under the Gran Sasso mountain, protected by 1,400 m of rock, reducing the flow of interfering particles by a factor of 1 million. The design of this multi-layered protection enables us to calculate the expected sensitivity of the detector during measurements. Despite all this, the dark matter should have no problem passing through the mountain to the detector, as it is only supposed to interact weakly with matter. The collision could therefore happen anywhere in the instrument, unlike radioactive particles which hardly run a few centimetres in matter and are concentrated around the walls of the detector. So, to address the challenge of detecting dark matter particles, we have an experiment with a target of massive nuclei, xenon, huge in size to increase the possibility of a collision, and sufficiently stable to collect data over a whole year. Above all, our experimental apparatus can measure the position of the interaction in the three dimensions of space.
And crucially, we can tell if the interaction occurred with the nucleus of the xenon atom (as a WIMP would) or with the atom’s electrons (as a parasitic photon would). Everything is ready to succeed in capturing the excess signal produced by a WIMP over the background noise. How does detection occur in the heart of the experiment? When a particle interacts in the detector, the impact causes a xenon nucleus (or electrons) to recoil. Two separate signals are thus produced. An initial scintillation signal is emitted a few nanoseconds after the interaction. These photons emitted in the ultraviolet are detected by photomultipliers that cover the top and bottom of the chamber. At the same moment, electrons, disturbed by the collision, move from the point of interaction along a strong, vertical magnetic field (80 V/cm) applied across the whole instrument. Arriving at the top, the electrons pass through the gaseous xenon by means of a stronger electrical field (10 kV/cm). They are reaccelerated and create a second scintillation signal. The position of the interaction in the perpendicular plane is deduced by the shape of the light impression left by this second signal on the photomultipliers. The time difference between the emission of the two signals and the speed of propagation of the electrons can be used to find the position along the vertical axis. The resolutions achieved in the three dimensions are better than 1 cm. Furthermore, the ratio between the intensities of the second and first scintillation signals are greater for electronic recoils than for nuclear recoils, enabling the nature of the particle which passed through the detector to be better determined.
THE HUNT CONTINUES
The last Xenon 1T measurement campaign lasted 278 days, over the 16 months from November 2016 to February 2018. The results of the analysis, with the lowest ever background achieved for a dark matter experiment, shows no excess particles. The data observed are therefore compatible with the expected radioactive background noise. We have not discovered dark matter yet. Despite this, this is a major result for particle physicists as it places limits on the mass of the WIMP and on its cross-section, i.e. the probability of its interaction with ordinary matter. These parameters, combined with other direct or indirect detection attempts, or attempts to produce dark matter in the LHC, have undermined part of one of the simplest theories, the minimal supersymmetry model. Other models remain perfectly feasible however.
The field is open and future experiments are already in the pipeline. Liquid xenon remains the main ingredient in the search for the WIMP. To be even more sensitive, Xenon 1T will grow to form Xenon nT, with 7 tonnes of xenon; its American version, LZ, is also under construction with 10 tonnes announced. China is also preparing PandaX-xT, with 6 tonnes of xenon. Progress in the technology is so fast that one order of magnitude of sensitivity is gained every three years. The future Darwin (comprising 40 tonnes) is still being discussed and initial tests are being studied.
The hunt for the WIMP will continue! Discovering it would enable a complete and coherent picture of how the universe works to be drawn up. However, if it remains elusive, a new challenge opens up for scientists - to rethink and theorise our understanding of the universe.
(*) Gamma rays are comprised of high-energy photons. (**) A nucleon is what makes up the nucleus of an atom, i.e. a proton or a neutron. Consisting of three quarks, it belongs to the family of baryons.
HAVE WE ALREADY DETECTED DARK MATTER ?
Last March, scientists at the Dama/Libra project at the Gran Sasso National Laboratory in Italy, announced they had observed a seasonal oscillation in the number of nuclear recoils measured in their crystals of thallium-doped sodium iodide. They attribute this variation to the flow of WIMPs passing through the Earth. It is not the first announcement by this project. Combined, the twenty years of results from this project are quite convincing. However, there is nothing to indicate it is dark matter. Even if the period of oscillation (365 days) and its maximum (beginning of June) agree with what dark matter would produce, the distribution of the nuclear recoils according to energy is not as expected. Moreover, Dama cannot differentiate between electronic and nuclear recoils. The origin of the fluctuation cannot therefore be identified. We are indeed bathed in a seasonally changing environment; what if one of these changes had not be taken into account in the measurement? Also, no other instrument is able to reproduce these observations, although they are supposedly more sensitive. To eliminate any doubt, the experiment must be reproduced independently using the same crystals. Cosine-100, in South Korea, has already started collecting data and the Sabre project is developing two experiments, one in Gran Sasso and the other in Australia.
WHAT ABOUT THE AXION?
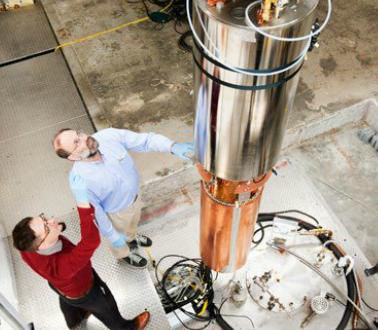
The axion is the main competitor particle of the WIMP for making up dark matter. Imagined at the end of the 1970s to solve an issue concerning the strong force (one of the four fundamental forces), the axion could be one million times lighter than the WIMP. There should therefore be a far greater quantity of them in the universe. Although the range of possible values for its mass and the probability of interaction with matter are high, experiments are hoping to detect it, such as ADMX at Washington University, which has just reach sufficient sensitivity to test the most probable models of axions. (Photo : The ADMX experiment at the University of Washington is testing axion models. Photo © Christian Foehr - 2013 University of Washington)
> AUTHOR
Julien Masbou
Astrophysicist
The particle astrophysicist Julien Masbou is a professor assistant. A scientist at Subatech laboratory (CNRS, Nantes University and Institut Mines-Télécom Atlantique), he hunts for particles of dark matter as part of the Xenon project.
Comments